By Rasoul Sorkhabi, Ph.D., CPG-11981
This article was originally published in The American Institute of Professional Geologists (AIPG), 2022
Dr. Rasoul Sorkhabi is a professor at the University of Utah’s Energy & Geoscience Institute, Salt Lake City.
Email: rsorkhabi@egi.utah.edu
Geoscience education, enrollment and employment are facing daunting challenges for a variety of reasons: (1) Competition from other sciences and fields such as biology, chemical engineering, business, and computer and information science; (2) public misconception of geologists as field workers (mostly men) hammering, digging or blasting rocks in rough and remote places or chasing earthquakes and volcanic eruptions; (3) insufficient efforts and outreach programs to educate the public and the youth about the significance and relevance of geoscience to life and society; (4) lack of modern courses around big questions and real issues to attract minds and monies; and (5) a sharp decline in employment by the oil and gas industry (Figure 1), which was traditionally a major employer of geology and geophysics graduates.
The modern world has been shaped by fossil fuels: coal, oil, and gas. These still constitute 80% of energy consumption (Figure 2) although renewables are and will be steadily increasing their shares. The burning of fossil fuels, which has steadily increased since the mid-19th century on par with growth in populations and industries, has increased the atmospheric carbon dioxide, a key culprit in the current global warming manifested in melting glaciers, floods, storms, droughts, and wildfires. Many rightfully fear that if we do not do something big and fast, these climate-driven phenomena will intensify and will be accompanied by sea level rise, inundation of islands and coastal cities (loss of land), ocean acidification, and habitat destruction for wildlife.
We are living in the midst of a major technological revolution in history – a transition to low-carbon industries, economies, and lifestyles. The energy transition is not going to be easy or fast. However, its challenges also come with real opportunities for innovation, research and development (R&D), new funds, and being part of the solutions.
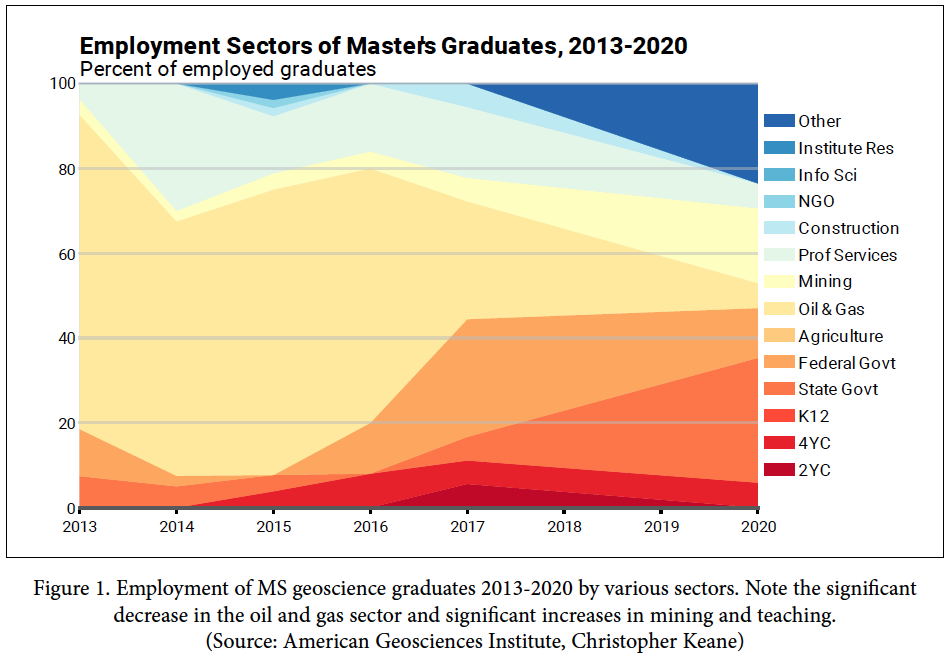
Carbon Capture and Storage/Sequestration (CCS) and Utilization (CCSU)
To avert the global warming catastrophe, it is necessary to reduce our emissions of carbon dioxide (and methane). It is also necessary to remove the extra CO2 from the atmosphere.
Carbon capture methods may be applied before (partial oxidization in a gasifier), during (the Oxy-fuel combustion with pure oxygen instead of air), or after the combustion process. Post-combustion carbon capture includes (1) direct air capture (DAC), which is less efficient because it requires considerable energy input to remove the dilute atmospheric CO2 (414 ppm), and (2) point source capture (PSC) from flue gasses at power stations and other industrial plants.
After CO2 is captured as a concentrated compressed stream, it needs to be transported and stored safely in underground rock formations, or utilized, for example, in producing chemicals such as methanol or injecting into oil fields for enhanced oil recovery (EOR). There are five mechanisms for geological trapping of CO2: (1) Structural trapping in a porous reservoir capped by an impermeable seal rock; (2) capillary trapping in the pore space of reservoir rocks; (3) solubility trapping by dissolution of CO2 into formation waters; (4) mineral trapping by reacting dissolved CO2 with fine-grained metal oxides (notably ultramafic mine tailings) to produce carbonates; and (5) biological trapping either naturally (tree plantation or reforestation) or artificially, for instance, fertilization of the oceans with iron to enhance phytoplankton activity and CO2 uptake.
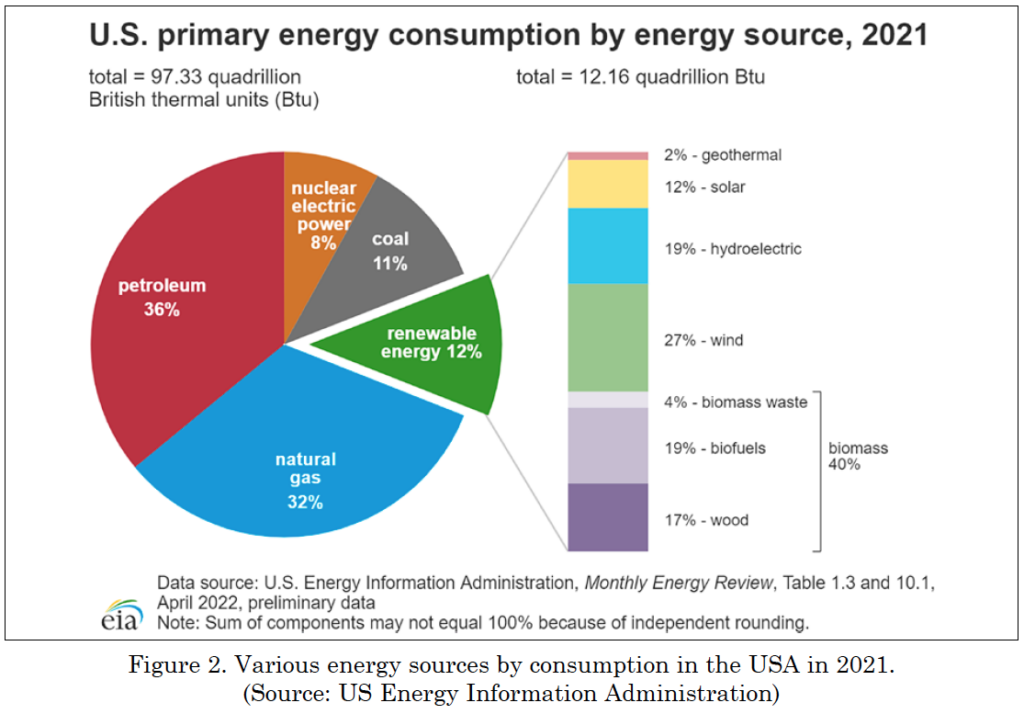
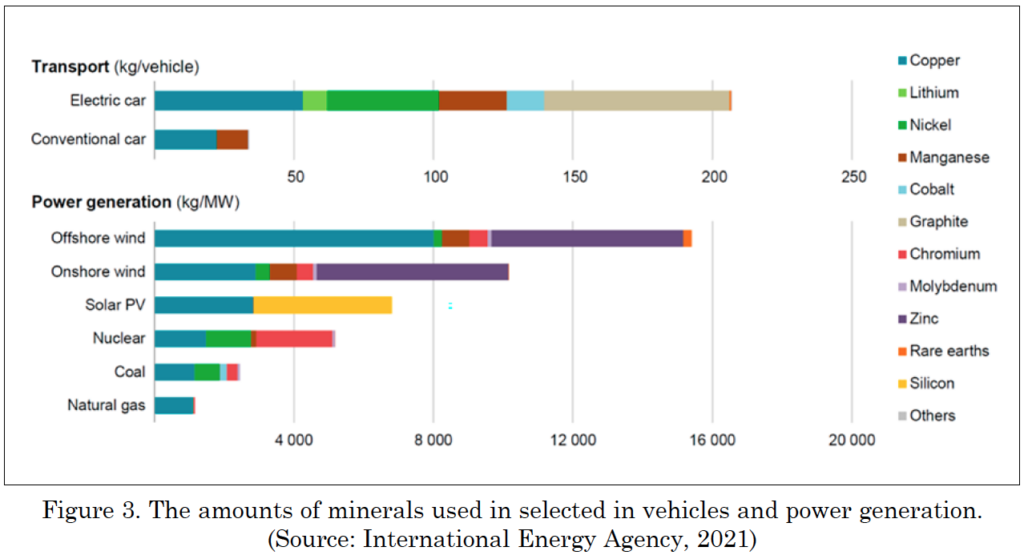
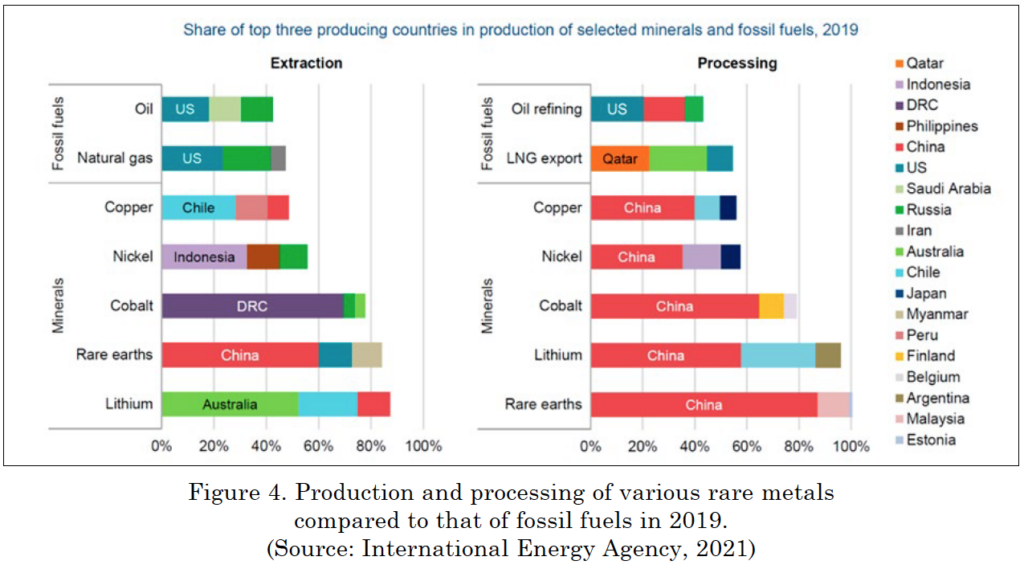
1. https://www.usgs.gov/news/national-news-release/us-geological-survey-releases-2022-list-critical-minerals.
2. IEA (2021) The Role of Critical Minerals in Clean Energy Transitions, Paris, 287 p.
The CCSU technologies are in their infancy; they are mostly on R&D laboratory or pilot demonstration scales, and have thus huge potentials for development. Many of the CCSU projects rely on engineering, but certain aspects require geoscientist’s skills. These include identifying and mapping the extent and size of underground storage sites, characterizing the petrophysical properties of potential reservoir rocks, seal and trap integrity, forecasting fluid-rock interactions, and geophysical monitoring of the CO2 storages. Underground carbon storage sites must be properly tested and selected; poor choices that result in CO2 leakages will undermine the public confidence in this promising solution.
The petroleum industry has long injected CO2 into subsurface reservoirs for EOR; the Permian Basin being the best example. The petroleum geoscientists’ skillsets would thus be highly desirable in managing the storage sites not only in active oil and gas fields for EOR, but also in abandoned fields where residual hydrocarbons would mitigate the carbonic acid formed from CO2 reaction with water.
Critical Minerals
Massive electrification of the world in the coming decades – electric vehicles, power plants and grids – will require enormous amounts of minerals, metals and rare-earth elements (REEs) (Figure 3). The US Geological Survey has identified 50 minerals and elements critical to the US economy, industries, and national security.1 European Union, Japan, and Australia have also assessed similar lists. These substances may not be geologically rare, but are in high demand and subject to high risks of supply disruptions as their production or processing are currently done in a handful of countries with China topping the list (Figure 4). A recent report by the International Energy Agency 2 suggests that global demand for lithium, cobalt, nickel, copper, and REEs will increase by 40-70% by 2040. The exploration, mapping, reserve estimates, and mining of rare metals and critical minerals offer research and funding opportunities for geoscientists.
Critical minerals and elements have either primary conventional sources (rocks and ores) or unconventional secondary sources from existing mines and their wastes such as coal fines and fly ashes, mine tailings, waste streams, smelter slag, brines, seawater deposits, and end-of-life productions. The latter category is currently receiving much attention as this would also help clean up the massive mining wastes accumulated for over a century. In addition, deep sea mining of manganese nodules, cobalt-rich crusts on seamounts, and sulfides from hydrothermal vents are investigated as new frontiers.
Geothermal Energy
With geothermal gradients ranging from 15 to 75 °C/km (mostly 30 ±10 °C/km), Earth is a gigantic heat machine, far greater than we can utilize or even imagine. Iceland sitting in an active volcanic terrain is a geothermal success story. Geothermal energy occurs in underground hot rocks and fluids either as dry steam (vapor-dominated) or wet steam (liquid dominated). A more detailed classification based on geological formation and temperature profile includes the following geothermal systems.
(1) Magmatic systems (between 700-1200 °C) at several km crustal depths, for which the exploitation technology has not been developed yet.
(2) Hot dry rock with temperatures of 180-240 °C in tight granitic rocks atop active magma chambers. Due to their impermeability, these rocks require fracture stimulation (with pressurized water or supercritical CO2) in order to create conduits for fluid circulation; therefore, they are also known as enhanced/engineered geothermal systems (EGS).
(3) Geopressured systems are formations with high temperature brines (170-180°C) in deep sedimentary basins. These brines can also be mined for their critical minerals and metals – a good example of “co production.”
(4) Hydrothermal systems include both hot water and vapor trapped in porous or fractured rock formations. Those with higher temperatures (>130 °C) are used for electric power generation, while lower water temperatures (<85°C) are directly used to heat buildings and greenhouses or provide hot spring resorts.
(5) Ground source heat pumps are the cheapest and easiest of geothermal systems as they utilize the ambient ground temperatures (10-20 °C at 2-3 m depths, depending on latitude) for winter-time space/water warming and summer-time space cooling through water circulation in high-density polyethylene tubes (closed or open loops).
Geothermal systems require heat and permeability; these rock properties should be mapped and quantified for field development. Geologists working on geothermal fields also construct 3D geologic models incorporating lithology, stratigraphy, structure, crustal depth and geothermal profile. Recently, attention has been given to abandoned oil and gas wells with high bottom-hole temperatures that can be retrofitted to geothermal power plants either as closed-loop (single well) or open-loop (injection and production wells) systems. In the UK, abandoned coal mines, which are located in many towns and are unaffected by seasonal temperature variations, are considered as a natural heat source.
Hydrogen
Hydrogen is a zero-carbon fuel burned with oxygen. It can be used in both fuel cells or internal combustion engines. Hydrogen vehicles are thus an attractive alternative to gasoline-powered vehicles. However, hydrogen does not occur naturally in large quantities on Earth; it should be “mined” from methane and coal (by reforming) or from water (by electrolysis). Depending on the processes used, various grades of hydrogen are identified3 (Figure 5), and these technologies need to be improved for better efficiency and minimal environmental impact.
Being the lightest element and a highly reactive gas, hydrogen is not easy to be stored. There are no natural hydrogen fields indicating that the subsurface geology does not provide a proven concept or analogue. Nevertheless, research is underway to examine if hydrogen can be stored in subsurface salt caverns.
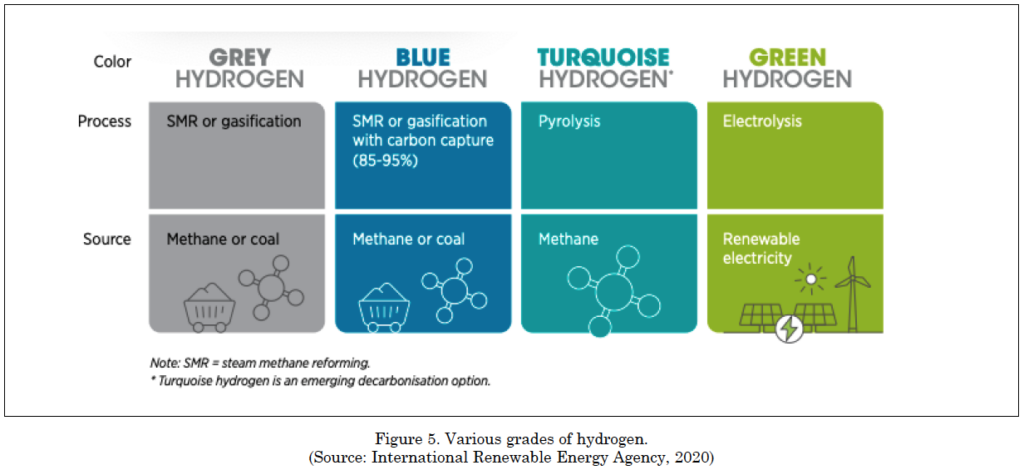
3. International Renewable Energy Agency (2020) Green Hydrogen: A Guide to Policy Making. Abu Dhabi, 57 p.
Energy Storage
The good news from Physics 101 is that energy comes in various types which can be converted from one to another. However, a key component of energy transition will be our capability to efficiently store energy for electricity not only in the short term (hours in the case of batteries) but also seasonally (months) where energy production and consumption from renewables (solar, wind, and geothermal) are localized. Various types of energy storage systems are undergoing significant R&D innovation and experimental improvements in terms of cost and benefit, size and capacity, efficiency (“roundtrip” energy loss effect), discharge (how long) and response (how fast) time, and so forth. Geoscientists can help identify and characterize underground formations or optimize earth materials for energy storage.
Natural Gas
Natural gas is the small brother of Big Oil. However, it emits respectively 50% and 25% less carbon dioxide than coal and crude oil4; moreover, natural gas is more abundant and is an established source of electric power. For these reasons, natural gas is often viewed as a bridge from today’s fossil fuel dominated civilization to a low-carbon world in the near future.
We witnessed the vital importance of natural gas supplies for energy security in early 2022 when Russia invaded Ukraine and threatened to cut its pipeline exports of natural gas to Europe, which has depended on Russian oil and gas for decades. For a foreseeable future, geoscientists will be needed to explore, discover and develop natural gas reservoirs in various sedimentary basins and regions of the world. Natural gas is a versatile resource: Dissolved gas and gas caps associated with crude oil, non-associated (free) gas fields, shale gas, coalbed methane, coal gasification, methane hydrates, synthetic gas, and even mantle volcanic gas. They all require various technologies. Liquefied natural gas (LNG) plants to transport this hydrocarbon fuel across the oceans as well as natural gas power plants will increasingly grow in the coming years.
Energy-Environment Nexus
Perhaps one of the most important subject areas for geoscientists is to investigate the impacts of energy sources, exploration, production, maintenance and consumption of various energy sources on the environment.
The public is well aware of the fossil fuels’ role in global warming; however, they are little aware of the environmental impacts of other energy technologies; for example, deep sea mining, wind turbine landfills, limited life of batteries, water availability for hot dry rock EGS, and so forth. In other words, no single energy technology today is all clean or all capable of meeting the demands of populations around the world. All types of energy sources and technologies need to evolve and improve to be efficient, abundant, and with minimum adverse impact on the environment. Environmental sustainability of energy technologies adds another dimension to R&D and careers in energy transition.
Towards New Geoscience Curriculums
With the exception of natural gas, which is an established industry, the above-mentioned subject areas are at the forefronts of R&D with funds in hundreds of billions of dollars offered by the US Department of Energy, Department of Defense, National Science Foundation, Canadian Office of Energy Research and Development, European Commission, European Research Council, UK Research and Innovation, as well as major foundations and corporations. These subject areas are yet to be incorporated in new geoscience curriculums in universities.
The geoscience community should embrace the current energy revolution in their teaching and training, and in doing so, two important trends need to be considered: Firstly, many of the research grants related to energy transition require collaborations between geoscientists and engineers. This means designing team-teaching “geoengineering” courses, assignments and research work in order to train graduates comfortable with data science, modeling, and working with professionals in the entire value chain of upstream (exploration and production) and downstream (distribution and marketing) processes. Secondly, a large number of the energy transition ventures are conducted by start-up entrepreneurial companies. Therefore, geoscience students, in their training, need to be exposed to business practices and economic knowledge base.
Information Sources
In recent years, various professional organizations have offered conferences and publications on how geoscientists can be part of the coming energy transition. These are great sources for creating educational materials on energy transition by universities and institutions. For more information visit their websites and publications.
(1) American Association of Petroleum Geologists (AAPG) publishes the monthly AAPG Explorer and organizes annual meetings including The AAPG Energy Transition Forum, held so far, in September 2018 (Amsterdam), October 2019 (Edinburgh), and May 2022 (London).
(2) Society for Exploration Geophysics (SEG) publishes the monthly Leading Edge, and since 2020 SEG/AAPG have organized IMAGE conventions where special sessions and panel discussions on energy transition and geoscientist workforce have been held.
(3) European Association of Geoscientists and Engineers (EAGE) publishes the monthly First Break, and since 2020 it has organized the annual EAGE Global Energy Transition & Exhibition every November in The Hague.
(4) The Geological Society, London, has organized a series of webinars on Geosciences and Energy Transition during 2021-2022, and in May 2022 it hosted a two-day conference in its headquarters (Burlington House) in London.
(5) GEO ExPro, a bimonthly magazine published in Europe, often includes articles on energy transition, renewable energies, and industry issues.
(6) Energy Transitions Commission is a global coalition of energy industry experts advocating the net-zero emissions; their website offers many informative reports and analyses.
4. “Carbon dioxide emissions coefficients by fuel,” https://www.eia.gov/environment/emissions/co2_vol_mass.php.